3D-printed calcium magnesium silicates: A mini-review
- 1 Polymer and Color Engineering Department, Amirkabir University of Technology, Tehran, Iran
- 2 School of Nano Science and Technology, Indian Institute of Technology, Kharagpur, West Bengal-721302, India
- 3 Polymer and color Engineering department, Amirkabir University of Technology, Tehran, Iran
Abstract
Calcium magnesium silicates (CMS) represent a class of minerals with diverse applications in fields ranging from geology to materials science. With the advent of additive manufacturing technologies, particularly 3D printing, novel opportunities have emerged for the synthesis and utilization of CMS-based materials. In this mini-review, we provide a thorough overview of recent advancements in the 3D printing of CMS compounds, including diopside (DPS), bredigite (BR), and akermanite (AKT). We discuss the synthesis methods, properties, and potential applications of 3D-printed CMS materials, with a focus on their role in biomedical applications. Furthermore, we highlight challenges and prospects in the field, emphasizing the importance of continued research and innovation in harnessing the full potential of 3D-printed CMS materials.
Downloads
References
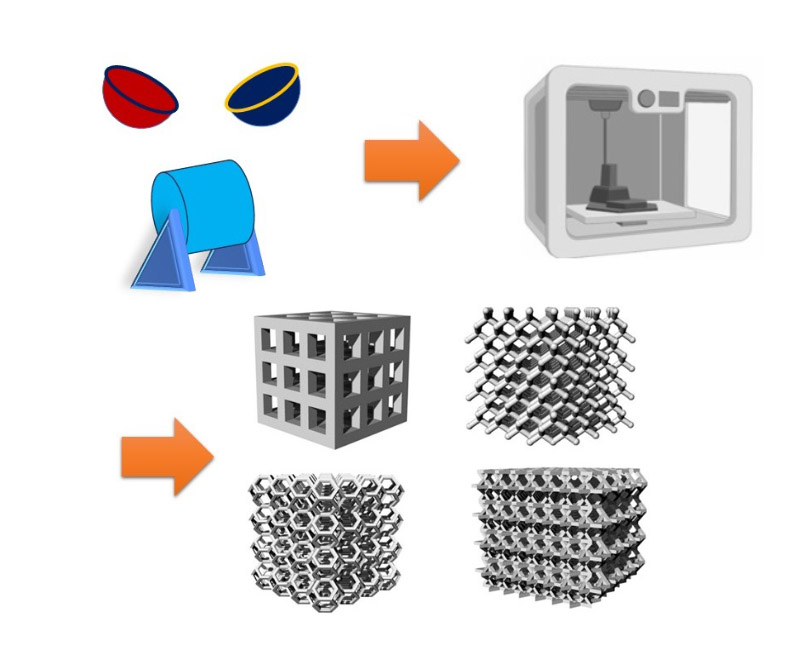
Copyright (c) 2024 Aidin Doroudi, Preeti Lata Mahapatra, Fatemeh Bakhshi

This work is licensed under a Creative Commons Attribution 4.0 International License.
Copyright
Authors are the copyright holders of their published papers in Synthesis and Sintering, which are simultaneously licensed under a Creative Commons Attribution 4.0 International License. The full details of the license are available at https://creativecommons.org/licenses/by/4.0/.
All papers published open access will be immediately and permanently free for everyone to read, download, copy, distribute, print, search, link to the full-text of papers, crawl them for indexing, pass them as data to software, or use them for any other lawful purpose without any registration obstacles or subscription fees.